Molecular Mechanisms of Photosynthesis. Robert E. Blankenship
Чтение книги онлайн.
Читать онлайн книгу Molecular Mechanisms of Photosynthesis - Robert E. Blankenship страница 25
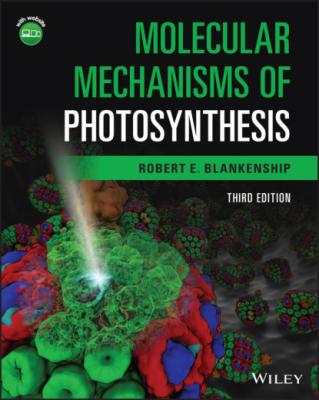
This disagreement may seem to be only an academic issue, but the outcome was essential to the development of a deeper understanding of the underlying chemical mechanism of photosynthesis. The discussion really boils down to energetics. The energy content of the three photons that Warburg thought were all that was needed is just barely enough to account for the free energy difference between the reactants and the products (see Chapter 13). Warburg was pleased with this result, which coincided with his nineteenth‐century romantic view of nature, summarized by the comment often attributed to him: “In a perfect world photosynthesis must be perfect.” Emerson's view was more practical, and thousands of subsequent measurements in many laboratories have supported his higher numbers for the quantum requirement for photosynthesis.
Exactly why Warburg obtained the results he did is still not entirely clear, but it is thought to have to do with interactions of photosynthesis and respiration, including transient “gushes” and “gulps.” The measurement shows only net oxygen production; to get the rate of photosynthesis, it is necessary to correct for the rate of respiration. If the rate of respiration is unchanged between light and dark, this correction will be accurate; but if photosynthesis inhibits respiration (as some modern evidence suggests), the correction will lead to erroneously low values for the quantum requirement. In retrospect, it is clear that Warburg, despite being a brilliant experimenter and very experienced professional scientist of the highest rank, fell into the very human trap of thinking that he knew what the answer should be and then not being sufficiently objective in evaluating his own experiments.
Unfortunately, the quantum requirement controversy took up the enormous time and effort of many of the foremost scientists of the day and didn't directly lead to a new understanding of the mechanism of photosynthesis. However, in the process of thoroughly examining the conditions required for the measurement of the quantum requirement for photosynthesis, some important new discoveries were made, which ultimately did lead to a much deeper understanding. Chief among these were the phenomena known as the “red drop” and “enhancement.”
3.10 The red drop and the Emerson enhancement effect
As part of his attempt to settle the controversy with Warburg, Emerson and coworkers made careful measurements in the 1940s of the quantum requirements for photosynthesis as a function of wavelength and obtained a most remarkable result. As the wavelength of light utilized for the experiment approached the red edge of the absorption of the chlorophyll, the quantum requirement went up dramatically. The action spectrum for photosynthesis is remarkably congruent with the absorption spectrum throughout much of the visible wavelength range, but drops off more quickly in this far‐red region (Fig. 3.4). An action spectrum is a plot of the effectiveness of light to cause a given effect, in this case oxygen evolution, versus the wavelength of light. This decrease in quantum yield (the reciprocal of the quantum requirement) at long wavelengths came to be known as the “red drop.” The interpretation of the red drop is that those chlorophyll molecules that absorb light at the extreme red edge of the absorption band do not do photosynthesis as efficiently as the chlorophylls that absorb light of shorter wavelengths. The long‐wavelength chlorophylls somehow behaved differently. Other measurements of photosynthesis and chlorophyll fluorescence in red algae, organisms that contain an antenna complex known as a phycobilisome, also suggested that the long‐wavelength chlorophylls were somehow inactive in photosynthesis. The red drop result was easily reproduced, but the significance of it was not understood until later.
However, the result of another experiment by Emerson and coworkers was even more bizarre (Emerson et al., 1957). He found that if the ineffective long‐wavelength light was supplemented with shorter‐wavelength light, it suddenly became capable of driving photosynthesis at good rates. A sample of algae was illuminated with red light, and the intensity adjusted to give a particular rate of O2 production, measured as always using a manometer. This light was then turned off, and a second light source, this time the inefficient far‐red light, was directed on the sample. The intensity of this light was adjusted to give a rate of O2 production comparable to that of the red light. This required that the intensity of the far‐red light be increased significantly, as expected from the earlier experiments that had shown its weak effect. The remarkable result was that, when both beams of light were directed on the sample at the same time, the rate of O2 production was greatly increased and was much higher than the sum of the two individual rates! This result came to be known as the enhancement effect, because of the enhancing effect of the short‐wavelength light. Additional experiments by Jack Myers and Stacey French (1960) showed that enhancement worked even when the two beams of light were not present at exactly the same time. These results made no sense in the context of the 1950s understanding of the mechanism of photosynthesis. Several years went by before a reasonable explanation was proposed for these and other puzzling results.
Figure 3.4 Absorption spectrum of chloroplasts (dashed line) and action spectrum for photosynthesis (dotted line). The red drop in the quantum yield of photosynthesis (solid line).
3.11 Antagonistic effects
A final experiment that pointed the way to the existence of two distinct photochemical systems working in series in photosynthetic organisms was carried out by Louis Duysens and coworkers from the Netherlands (Duysens et al., 1961). Duysens was a pioneer in developing sensitive spectrophotometric methods to monitor photosynthetic systems. The experiment was to measure the oxidation–reduction state of cytochrome f in the sample upon illumination using various wavelengths of light. When the cytochrome is reduced, the absorbance spectrum changes, permitting quantitative measurements of its redox state (Fig. 3.5). Duysens found that far‐red light caused the cytochrome to become oxidized, whereas shorter‐wavelength light caused it to become reduced. The two colors of light had opposite, or antagonistic, effects. A particularly clear effect was observed using the red alga Porphyridium cruentum, which has phycobilisome antenna complexes. The effects are easily observed with this organism, because, as we now know, the phycobilisome antenna complex preferentially directs excitations mostly to one of the two photosystems. As is often the case, the choice of the experimental system in which an effect is emphasized was important to the initial understanding of the effect. Subsequent measurements have shown that the effect is, of course, a general one, observed in all oxygen‐evolving photosynthetic organisms.
Figure 3.5 Antagonistic effects on cytochrome oxidation. Irradiation with the light of one color causes the cytochrome to become more oxidized, while irradiation