Molecular Mechanisms of Photosynthesis. Robert E. Blankenship
Чтение книги онлайн.
Читать онлайн книгу Molecular Mechanisms of Photosynthesis - Robert E. Blankenship страница 26
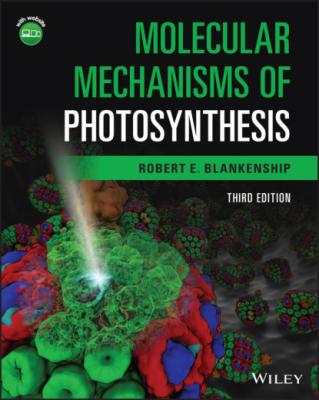
Source: Duysens et al. (1961)/Springer Nature.
3.12 Early formulations of the Z scheme for photosynthesis
All these experiments (and some others not discussed here) suddenly crystallized into a consistent formulation for photosynthesis about 1960. Robin Hill and Fay Bendall published a short paper in Nature in 1960 outlining the concept of two sequential photochemical systems arranged in tandem, so that the products of one system became the substrates of the other system (Hill and Bendall, 1960). Their formulation was based primarily on the observation that the redox potentials for two cytochromes found in chloroplasts were intermediate between the potentials of both the reductant (H2O) and the oxidant (NADP+) involved in photosynthesis. In order for them to be participants in the light‐driven electron flow, it was necessary to propose two photochemical processes and an energetically downhill intermediate step. Their original scheme is shown in Fig. 3.6. It has come to be known as the Z (for zigzag) scheme of photosynthesis (Govindjee and Björn, 2017).
Figure 3.6 Hill and Bendall's (1960) original formulation of the Z scheme for photosynthesis. Modern Z schemes are plotted with the y‐axis reversed in sign, so that more reducing species are at the top of the diagram and more oxidizing species are at the bottom. The action of light is shown as a vertical arrow in which a relatively highly oxidizing species is converted to a highly reducing one by the action of light.
Source: Hill and Bendall (1960) (p. 137)/Springer Nature.
The slightly later publication by Duysens and coworkers provided the crucial experimental evidence for this proposal, in the form of the antagonistic effects described above. One photochemical reaction, now known as Photosystem II, oxidizes water and reduces cytochrome f, while the other, now known as Photosystem I, oxidizes cytochrome f and reduces NADP+ (Duysens et al., 1961; Duysens, 1989).
The puzzling results of the red drop and enhancement effects are easily explained by this formulation of two photochemical reactions connected in series, if the absorption spectra of the pigments that feed energy to them are not quite the same. The shorter‐wavelength chlorophylls (and the phycobilisome antennas in those organisms that contain them) preferentially drive Photosystem II, whereas the longer‐wavelength pigments preferentially drive Photosystem I. Optimum rates of photosynthesis are observed when both short and long wavelengths are present, as found in the enhancement experiments. The light absorbed by the long‐wavelength pigments does not have enough energy to drive Photosystem II, so the entire system grinds to a halt if only far‐red light is used, thus explaining the red drop phenomenon. In retrospect, it was fortunate that the two photosystems had somewhat different wavelength optima, as the key early experiments demonstrating their existence all relied on preferential excitation of one or the other photosystem by carefully selecting the illumination regime.
The series formulation for oxygen‐evolving photosynthesis has been tested and questioned many times since 1960 and has withstood all challenges. There is now no doubt that this basic framework for photosynthetic electron flow in oxygen‐evolving organisms is correct. As we will see in later chapters, the two photosystems have been separated, biochemically purified, and their structures determined. The proteins that make them up to have been identified, and the genes that code for them identified and sequenced. While many questions remain about how the two photosystems interact and how both energy input to them from the antennas and electron flow between them is regulated, the Z scheme is an essential feature of the modern understanding of photosynthesis.
Of course, there are some types of photosynthetic organisms that contain chlorophyll‐like pigments and exhibit light‐dependent growth, but do not evolve oxygen. We encountered them earlier in our discussion of the van Niel formulation for the redox nature of photosynthesis. These anoxygenic (non‐oxygen evolving) photosynthetic organisms do not display any of the enhancement or red drop effects described above that led to the discovery of two photosystems in oxygen‐evolving organisms, which suggests that they contain only a single type of photosystem. Biochemical and genetic studies confirm that these organisms, which are all bacteria, indeed contain only a single type of photosystem. These simpler photosynthetic organisms have been essential for learning about the basic chemical mechanisms of photosynthesis. These organisms are discussed in detail in Chapter 6. They also provide clues to how the complex two‐photosystem version of photosynthesis found in more advanced organisms may have arisen and evolved. This is discussed in Chapter 12.
3.13 ATP formation
So far, we have focused first on the overall process of photosynthesis and then on the discoveries leading up to the discovery of the series formulation of electron transport in oxygen‐evolving photosynthetic organisms, resulting in the reduction of NADP+ to NADPH. There is another critical part to the story, however, involving the light‐dependent formation of ATP and the subsequent utilization of these two products to reduce CO2 to carbohydrates. The discoveries of these processes paralleled the discoveries of the electron transfer processes.
The discovery that chloroplasts could make ATP in a light‐dependent manner was made in 1954, by Daniel Arnon and coworkers at the University of California, Berkeley (Arnon et al., 1954). The idea that chloroplasts could make ATP, in a process called photophosphorylation, initially met with considerable resistance, because it was well known that mitochondria produced large amounts of ATP and, since chloroplasts in many ways drive the mitochondrial reaction in the opposite direction, this initially seemed backward (Arnon, 1984). An analogous discovery of light‐driven ATP formation in non‐oxygen‐evolving purple bacteria was made by Howard Gest and Martin Kamen (1948). The chemiosmotic hypothesis, the theoretical framework for the mechanism of how photon energy is stored in ATP, was provided by the incisive analysis of Peter Mitchell in the 1960s and 1970s, for which he received the Nobel prize in 1978 (Mitchell, 1979). We will discuss the details of the ATP synthesis process in Chapter 8.
3.14 Carbon fixation
At approximately the same time as Arnon was demonstrating photophosphorylation on one side of the Berkeley campus of the University of California, Melvin Calvin, Andrew Benson, and coworkers were working to understand the details of the carbon assimilation process itself on the other side of the campus (Calvin, 1989; Benson, 2002). They elucidated the chemical reactions that convert CO2 and assimilatory power into carbohydrates. These reactions have become known as the Calvin–Benson cycle, and Calvin was awarded the Nobel Prize for chemistry in 1961 in recognition of the brilliant elucidation of this complex set of reactions. He and his coworkers used the newly developed method of radioactive tracers, injecting algae with 14CO2 and then following the path of the radioactivity in the products (Creager, 2013). We will discuss the details of the Calvin–Benson cycle and other aspects of carbon metabolism in Chapter 9.
References
1 Arnon, D. I. (1984) The discovery of photosynthetic phosphorylation. Trends in Biochemical